Saving our Skins: Chapter 3. Changing of the Guard
Atmospheric Reflections from a Lauder Stargazer
A seismic shift in the work at Lauder. The first version of this chapter was posted at Substack on the 40th anniversary of my arrival at Lauder.
Updated September 4, 2021
Lauder’s research emphasis was slowly starting to shift. Long-range communications no longer relied on atmospheric reflections, and instead were starting to use shorter wavelength signals bounced off satellites. And navigation too had moved on, with the advent of GPS navigation systems.
The two main motivations for our upper atmospheric research had been torpedoed. The money began to dry up and interest waned.
Fortuitously, a few months before my arrival another visitor, Tony Harrison, from the University of Calgary in Canada, had visited Lauder to make the first upper-atmosphere measurements of nitrogen dioxide (NO2) in the southern hemisphere. This was in response to another perceived threat to ozone – a threat from jet aircraft flying at the same altitudes. Most atmospheric ozone is in the stratosphere, a region of the atmosphere that extends from around 10 km up to about 50 km, with peak ozone amounts occurring near 25 km altitude. Much higher than the clouds and weather, but much lower than the aurora.
The two main atmospheric gases are nitrogen (N2, 78 percent) and oxygen (O2, 21 percent). Together they account for 99 percent of its mass, although trace amounts of the oxides of nitrogen are always present and contribute to the natural balance of ozone in the atmosphere. But, at extreme temperatures, such as in the internal combustion engine, these gases can combine directly to form more nitric oxide (NO), which can destroy more ozone. That would disturb the natural balance. Up to the 1970s that hadn’t posed a problem because flight altitudes were well below the ozone layer. A notable exception was on polar routes (mainly over the North Pole), where the ozone layer is lower and can extend down below the 10 km flight altitude. There was a recognised increased risk of ozone poisoning for passengers on those flights.
But the risk to the ozone layer changed with the introduction of the new supersonic Concord jet, a joint venture between France and the UK. The first scheduled services had begun a couple of years earlier, in 1976, and they were the expected future for intercontinental travel. (The expected fleet never eventuated, but for economic reasons rather than because of concerns about the effect on ozone.) These new faster jets would fly much higher where there is less air resistance, so fuel efficiency is increased. Harold Johnston, a chemist from the University of California, had recently suggested that nitrogen oxides emitted from their exhausts could destroy ozone. The chain of chemical reactions involved is called a “catalytic cycle”, where tiny amounts of the catalyst gas, nitric oxide in this case, are responsible for the destruction of many molecules of ozone.
The reaction cycle is initiated by nitric oxide (NO) reacting with ozone (O3) to form nitrogen dioxide (NO2) and an ordinary oxygen molecule (O2). The NO2 so formed then reacts with an atomic oxygen atom (O), to reform nitric oxide (NO) and molecular oxygen (O2). There needs to be a ready supply of atomic oxygen for the cycle to proceed, but that is always the case at these altitudes during daylight hours, being produced by UV radiation from the Sun breaking apart molecules of ozone or NO2. The net effect of these steps is the conversion of ozone and atomic oxygen to two oxygen molecules (O3 + O → 2O2), just a reconfiguration of 4 oxygen atoms with no effect on NO in the process. The chain reaction continues because a product of the reaction sequence is another molecule of NO, which can in turn deplete ozone, and so on. So a single molecule of NO can destroy thousands of ozone molecules before being consumed in some other reaction.
It’s hard to measure atmospheric NO, but by measuring nitrogen dioxide, you get an indirect handle on how much of it there is, and how important the cycle could be. Paul Johnston and I further developed Harrison’s method to measure both NO2 and O3 in the stratosphere. Paul was – and still is – the technical whiz kid. And a great mentor. This was the beginning of our switch from upper atmosphere (ionospheric) research to middle atmosphere (stratospheric) research. The measurement method is called “Differential Optical Absorption Spectroscopy” (DOAS), a method which is now widely used throughout the world’s atmospheric science community.
Our initial measurements of NO2, which started in 1980, showed that its concentrations over Lauder have strong and repeatable seasonal and diurnal patterns, which demonstrate that its abundance is dominated by photochemistry initiated by sunlight. We use the term “abundance”, but it’s a bit of a misnomer because the amount of NO2 in the atmosphere represents only 0.1 parts per billion of the total gas concentrations. Even where its concentration peaks in the stratosphere (and plays an important role in ozone chemistry), it’s still only a few parts per billion.
In the spring of 1981, we extended the measurements to Antarctica. I was to have taken the instrument down on one of the annual winter resupply flights (called the WinFly operation) in August, but I had to defer that responsibility to one of my colleagues because our third son Hamish was born on August 17. I wasn’t too disappointed about missing out on the trip to Antarctica, as I presumed there would be plenty more chances. But I was wrong. It never happened.
The measurement method is one of many examples of remote sensing undertaken at Lauder. It involves taking spectra of sunlight scattered from the zenith sky (i.e., from directly overhead) at sunrise and sunset. The absorption bands used are in the violet part of the spectrum, at wavelengths around 435 nm. At twilight, the light-path through the stratosphere is about 20 times longer than for overhead Sun before scattering. But the light-path through the lower atmosphere after being scattered is much shorter. The method is therefore less sensitive to pollution in the lower atmosphere. We later went on to develop the method to measure water vapour and ozone. They weren’t the most accurate ozone measurements available, because of uncertainties in the light path, and because the absorption signatures were very small. In fact, our first publication sometimes showed negative amounts of ozone! Nevertheless, these were the first published measurements of ozone derived from absorptions at visible wavelengths (in the “Chappuis” absorption bands, named after their discoverer). Previous measurements had made use of the much stronger absorptions of ozone in the ultraviolet region.
We then shifted our emphasis briefly to measuring the same gases in the lower atmosphere, to see whether pollution could affect the stratospheric measurements.[1] We set up a powerful Hella Rallye car headlamp on the side of a barn about 10 km away and another halfway up the hills about 23 km away and looked for the absorptions by NO2 in the spectra after the light had traversed the path to the laboratory. It was challenging, because there were huge variations in intensity due to atmospheric turbulence.
You’ve seen the effects of turbulence - in the twinkling of stars. It’s not the stars themselves that twinkle. The twinkling is the intensity changes caused by tiny variations in air density in the Earth’s atmosphere which cause light to bend (refract). Interference between the slightly different light paths can result in constructive interference, where the star appears brighter, or destructive interference, where the star can nearly disappear momentarily. The sun and moon don’t seem to twinkle because of their larger angular extent. As seen by an observer on Earth, they each subtend an angle of about 0.5° from one side to the other. The light coming from each side therefore traverses a slightly different path through the atmosphere, so the effects of turbulence are smeared out. If you look carefully at the night sky, you can distinguish planets from stars from their twinkling. Although planets are much smaller than stars, they are so much closer that they subtend larger angles, and twinkle less.
For our distant light source, the intensity changes due to turbulence were tens of percent of the signal, while the spectral absorptions signature we were looking for were tiny – much smaller than 1 percent.
Our spectrometer took several seconds to scan the wavelength range where NO2 molecules absorbed, so the tiny signals from those NO2 absorptions were swamped by the turbulence effects. Paul came up with a neat way around the problem. He positioned a piece of glass in front of the spectrometer to deflect a small fraction of the light to another detector which responded to only the turbulence effects. The remaining beam went into the spectrometer which measured both the turbulence and the spectral absorption. He then took the ratio of the two signals, leaving just the spectral signal of interest. Very clever. Nowadays, it’s much easier, because modern instruments don’t have to scan the spectrum wavelength-by-wavelength using a single detector. Instead they use arrays of detectors (called diode arrays) to sample the whole spectrum simultaneously so the effects of turbulence disappear.
The technique was clever in another way too. In those days, the electronics to convert the analogue voltage signal to a digital value for logging by computer were more primitive. The converters available didn’t have enough precision to even see the tiny differences we were looking for. But we got around that by averaging for several minutes, taking advantage of the fact that the signal changed from other causes. We made use of the turbulence problem to improve the detection threshold. Using the ingenious technique, we showed that the air surrounding our laboratory sometimes had the lowest amounts of NO2 ever measured. Sometimes as tiny as 20 parts per trillion (ppt). At those times the air was as clean as anywhere on the planet. That confirmed that our twilight DOAS measurements really were of stratospheric NO2 and were therefore highly relevant to the ozone problem.
Because of the clean air over Lauder, the measurements of NO2 in the stratosphere above are the best in the world. They have continued to the present day, with only a small break when I was in the UK and parts of the instrument were pilfered for Antarctic measurements. Such was the shoe-string budget we were operating under. Luckily, we managed to quickly get replacement parts, and only a few months of data were lost. The long time-series has been invaluable for investigating atmospheric changes, including the effects of increasing greenhouse gases.
With the change in emphasis, the older upper atmosphere research programmes at Lauder were gradually wound down. That included the removal of an old 10-wheel open-cab GMC military truck that had been converted to a mobile optical observatory.
We sold it for $1 to a mechanically-gifted-retiree-scavenger. He was a friend of my dad and his name was Ian Coutts, or “Couttsy” to his mates. Like my dad, Couttsy was a keen glider pilot, who had his eye on the winch that was mounted above the front axle. The truck hadn’t been moved in years, so we were impressed when he managed to find that 4 of its tyres could still hold air. He replaced the battery, and somehow got the truck going. He then drove it on an epic cross-country journey to the gliding mecca of Omarama, via the Hawkdun Runs 4-wheel-drive track and the Mt St Bathans saddle; a trip that involved multiple river crossings with scoured approaches. At the Omarama air strip, he used its winch as part of a tow for launching gliders, or sailplanes as they’re sometimes called. He later renovated the truck into a collector’s item. Lauder’s association with Omarama would be rekindled two decades on. But that’s another story that I’ll delve into in chapter 19.
But I digress. The first deployment of our new NO2-measuring instrument to Antarctica was in the spring of 1981. It was set up at the Arrival Heights Laboratory near Scott Base (78°S), close to the slopes of Mt Erebus - the final resting place of flight NZ901.
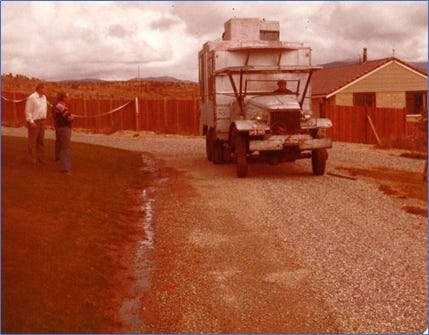
At that latitude, the twilight measurement method works only for the short seasonal transitional periods in spring and autumn when the Sun rises or sets. In summer it remains above the horizon all day, and in winter it remains continuously below the horizon during the long “polar night”.
Our first complete set of springtime data was in 1982. Just as at Lauder, the measurements showed that NO2 in the stratosphere is dominated by photochemical reactions driven by sunlight. The seasonal changes were like those at Lauder, but there were much larger short-term variations, depending on whether the air being sampled was from higher latitudes still within the polar night, or whether it had been exposed to sunlight at lower latitudes where photochemical reactions can occur.
Meanwhile, the problem that was brewing in Antarctica and would make Air New Zealand’s “Litany of Lies” look like a walk in the park was soon to be discovered. And our measurements there in 1982 showing that the amounts of stratospheric NO2 were similar in New Zealand and Antarctica were to prove hugely important.
Another two atmospheric chemists at the University of California, Sherry Rowland and his post-doc Mario Molina, had suggested that the ozone layer was under threat from a new class of chemicals called chlorofluorocarbons, (or, simply CFCs). The most abundant of these were CFC-11 and CFC-12. Their boiling and freezing points made them ideal as refrigerants. Unlike other refrigerants available, they were unreactive, and therefore much safer than the alternatives. This stability also made them useful as foam blowing agents (as used in the manufacture of materials like polystyrene), and as propellants for spray cans, which were becoming widely used in the cosmetic and construction industries. They sounded like the perfect solution - until it was realised that although they would never break down in the lower atmosphere, over a prolonged period they could mix into the stratosphere where they would be exposed to ultra-violet radiation that’s strong enough to break the molecules apart. The chlorine atoms released in that process could potentially destroy ozone in a series of catalytic cycles like that involving NO2. In this case, just one chlorine atom could end up destroying hundreds of thousands of ozone molecules.
Unlike the nitrogen oxides, these CFCs don’t occur naturally. They are manufactured exclusively by a small group of companies, including Du Pont and Allied Chemicals in USA. Despite mounting evidence that they might affect the ozone layer, the manufacturers were arguing strenuously. Their cash-cow chemicals could not be blamed, they said, so that no action was needed yet. It needed more research. And in the meantime, they should be able to continue to profit from their manufacture.
This was the set-up for one of the greatest battles in the history of politics and the atmospheric sciences. It was an issue that was to become an all-consuming focus of our research in the decades that followed.
[1] Much later, when concerns were raised about climate change issues from increasing greenhouse gases (GHGs), we returned to measuring the concentrations of a wide range of tropospheric gases, including the “big three”: carbon dioxide (CO2), methane (CH4) and nitrous oxide (N2O).
[1] Much later, when concerns were raised about climate change issues from increasing greenhouse gases (GHGs), we returned to measuring the concentrations of a wide range tropospheric gases, including the “big three” GHGs: carbon dioxide (CO2), methane (CH4) and nitrous oxide (N2O).
Next week: How Lauder became the world’s best site for measuring ozone.